Physics—Semester A
Comparing apples and forces.
- Credit Recovery Enabled
- Course Length: 18 weeks
- Course Type: Basic
- Category:
- Science
- High School
Schools and Districts: We offer customized programs that won't break the bank. Get a quote.

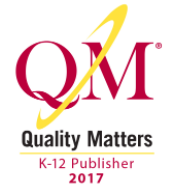
Shmoop's Physics course has been granted a-g certification, which means it has met the rigorous iNACOL Standards for Quality Online Courses and will now be honored as part of the requirements for admission into the University of California system.
This course has also been certified by Quality Matters, a trusted quality assurance organization that provides course review services to certify the quality of online and blended courses.
Shmoop's Physics course has been granted a-g certification, which means it has met the rigorous iNACOL Standards for Quality Online Courses and will now be honored as part of the requirements for admission into the University of California system.
When it comes to most high school classes, teachers can get away with saying, "Relax, it's not rocket science." Not so with this class.
Physics literally is rocket science. Well, at least an introduction to rocket science. We'll need a bit more knowledge before we're ready to build our own spaceship, but we'll be well on our way by the end of this course.
A lot of physics classes can be a bit dense and muddled up with all the math, but this course is different. For one thing, it's chock full of activities that put physics into perspective. After all, that's the big idea behind physics: to explain the world around us.
On top of that, the conceptual and math parts are presented with a focus on real-world scenarios. That means, in between building incline ramps and chucking medicine balls while riding a skateboard, we'll learn why parachutes keep skydivers from going splat, how to determine the acceleration of jets, and why Earth keeps circling the Sun rather than hurtling off into space.
Between the readings, practice problems, and activities, this Common Core-savvy course will have us ready to hobnob with NASA scientists and discuss the finer points of:
- Graphing objects in motion (so we can see where they've been)
- Predicting the motion of objects (so we know when to duck)
- How forces determine the motion of objects (and cause injuries)
- How gravity works, and what the deal is with circular motion
- How momentum and energy are tied together, and how both are conserved
- Why collisions are so gnarly, and why something as simple as crumple zones on a car can help protect us when they happen
So why wait? Launch into physics, and when someone starts bugging you, you can kindly tell them to move along because you're training to become a rocket scientist.
P.S. Physics is a two-semester course, and you're looking at the breakdown for Semester A. Go check out Semester B by clicky-clicking here.
Technology Requirements
From a technology standpoint, all you need for this course is a web browser-capable computer and a reliable internet connection. A tablet works, too, if you don't mind typing on it. Additionally, access to a scanner or digital camera, or a cellphone with a camera, or jeez, even a webcam, will come in handy, since you'll occasionally need to upload images of diagrams you draw. That's it.
Required Skills
Knowledge of Algebra concepts
Unit Breakdown
1 Physics—Semester A - Introduction to Physics
You can't build a house if you don't know how to use a hammer and a saw. Same deal with physics, but instead of a hammer and a saw, physicists use measurements, graphs, variables, and a fair amount of trig and algebra. This unit is basic training for physics to make sure no one smashes their thumb with a hammer. Figuratively speaking, of course.
2 Physics—Semester A - Constant Velocity Motion
It turns out there's a difference between distance and displacement. Same deal for speed and velocity. We'll learn what those differences are and, more importantly, why we should care about those differences. Once we've got that in the bag, we'll learn how to measure and calculate velocity and position for all kinds of everyday scenarios. Ever wonder how long it would take an orangutan to walk a mile on its hands? No problem. We can figure that out.
3 Physics—Semester A - Uniform Acceleration
In real life, objects don't just move around at the same velocity twiddling their thumbs. Instead they tend to speed up and slow down, and that's what this unit is all about. We'll bust out some graphs, monkey around with gravity as a source of acceleration, and then master the equations for acceleration while exploring real world scenarios like drag racing and skydiving.
4 Physics—Semester A - Two-Dimensional Motion
It turns out humans are already masters of 2D motion. Think we're wrong? Throw a tater tot arcing up into the air in the general direction of a hungry teenager's mouth. Trust us, they'll do the rest and deftly snatch that tasty morsel out of the air with their slathering maw. This unit will show us how to calculate trajectories for things a bit more complex than tater tots.
5 Physics—Semester A - Newton's Laws
Sir Isaac Newton is sort of a big deal. In this unit we'll learn about his three laws of motion and how they explain the world of motion. To facilitate this, we'll be busting out our mad doodling skills to draw free body diagrams. This unit really brings together everything we've learned in the prior units, and by the end we'll be able to explain all varieties of motion around us, from someone taking a digger on the icy sidewalk to a monster truck tractor pull.
6 Physics—Semester A - Gravity and Circular Motion
Sometimes things don't keep moving along in a straight line. The planets come to mind, and so to do those spinny carnival rides that make Papa Shmoop hurl. In this unit, we'll learn how gravity works and why it causes celestial bodies to go in circles instead of drifting off like a kid in a candy store. We'll also learn about centrifugal force and centripetal force. Yes, they're different things.
7 Physics—Semester A - Momentum
Momentum is the mother of all funny wipeout videos. In this unit we'll learn the physics behind things like parkour fails and skateboard tricks gone wrong. On top of that, we'll learn about a little-known thing called impulse, which turns out to be the key to understanding how force and motion are intertwined. It also helps us understand collisions and how safety features on cars help save lives.
8 Physics—Semester A - Energy
Motion and momentum have a friend who goes everywhere they go: energy. Like momentum, energy is conserved. We'll go into more depth studying collisions, exploring both inelastic and elastic collisions. Then we'll revisit car collisions and bring energy into the equation when it comes to analyzing safety features. To add a good bit of fun into the mix, we'll get to tap into our inner mad scientists and create Rube Goldberg devices.
9 Physics—Semester A - Midterm Review
We've covered all kinds of formulas, equations, and out-there scenarios. It's enough to make your head swim laps in confusion. That's why we're gonna take some time to review everything we've worked on and help keep all this stuff straight.
Recommended prerequisites:
Sample Lesson - Introduction
Lesson 5.02: Newton's First Law
Newton's First Law of Motion, or inertia as it is commonly called, is pretty evident around us when it comes to objects that are not moving. Right? A burrito sitting at rest on a plate will continue to sit there unmoving. It's not magically going to fly into our mouth of its own accord, no matter how hungry we are. Newton's Second Law of Motion says a mouthward unbalanced force, such as one exerted by a hand, is needed to accelerate that burrito and get it moving in the direction of our mouths.
We see inertia every day in the world around us and pretty much take it for granted. Cars at a stop light will stay at rest until the engine exerts a net force on the axles and gets the wheels moving. The junk on our bedroom floor will stay there unless we exert a net force to put it away, and the lint in our belly buttons is staying put unless we clean it out. Gross.
What about the other side of Newton's First Law, though? An object in motion with no net force on it will stay in motion. We don't see that in the real world very often, if at all. Instead, it seems that objects in motion tend to slow down and come to a stop. Even on a skateboard or razor scooter, we need to keep pushing ourselves along. What gives? Is Newton's First Law a bunch of bologna?
Not at all. It just so happens that there are all sorts of forces acting on objects in motion in the real world, chief among them, friction. Friction, like gravity, is an invisible force, so it's not super obvious to the casual observer. That's why it took Shmoopy smart people like Galileo and Newton to put the pieces of inertia together.
In this lesson, we'll explore Newton's First Law of Motion in more depth and talk about the everyday forces that sneakily use Newton's Second Law to slow down objects in motion. The only difficulty here will be overcoming our own inertia.
Sample Lesson - Reading
Reading 5.5.02: Why All the Friction?
We know the basics of Newton's First Law of Motion, but let's delve a little deeper. There is a very specific criterion for the first law to be in effect: there has to be zero net force acting on an object.
This can happen in one of two ways. There can either be no force acting on an object, or there can be balanced forces acting on an object that cancel each other out. The second scenario is the more common of the two because every object that has a mass is always being acted on by the force of gravity, whether it is at rest or in motion.
Take our pal Isaac pictured above. Gravity is pulling him toward the center of the Earth, but he's not accelerating toward the center of Earth. Why? Because the chair is holding his butt up. The chair holding Isaac up slightly deforms and exerts a force perpendicular to its surface (what we call a normal force) on Isaac. In this case, where the surface is flat, the normal force is equal in magnitude and opposite in direction to the force gravity is exerting downward on Isaac. As such, the forces balance out to a net of 0 N. Newton isn't accelerating anywhere unless some new unbalanced force acts on him.
This is the case for pretty much all objects at rest. Objects in motion follow the exact same principle. If the net force exerted on an object is zero, then it will keep moving with the same velocity. In either case, the acceleration stays at zero. As you may recall, acceleration is a change in velocity, which includes both speed and direction.
As we can see in the diagram above, an object at rest experiences no acceleration if the forces are balanced, meaning the object stays at rest. Meanwhile, an object in motion also experiences no acceleration if the forces are balanced, meaning the object keeps cruising along at the same speed in the same direction.
Who Put on the Brakes?
In real life, objects in motion are rarely subjected to balanced forces, at least naturally. This is why they experience acceleration in one way or another; they're being subjected to invisible, unbalanced forces. What are the mysterious sources of these unbalanced forces? We've already had a lot of experience with one of them: gravity.
If there's nothing to hold an object up, gravity will cause it to go into free fall toward the center of the Earth with an acceleration of 9.8 m/s2. We did lots and lots of vector trajectory calculations in the previous unit that were subject to gravity, so we're no stranger to it.
The other invisible source of unbalanced force is friction. Friction is the force exerted by one object on another as the two objects slide across one another. Depending on the surfaces, the force of friction will vary.
Ever try sliding across a hard floor on your socks, or at least seen Tom Cruise do it in Risky Business? It works pretty well because the friction between socks and a hard floor is relatively low. Even so, you still slow down and come to a stop due to friction. Even Tom Cruise comes to a stop and he's a movie star.
If Mr. Cruise were to try the same stunt wearing sneakers instead of socks, what would happen? Well, let's just say he wouldn't cruise very far. That's because the friction between rubber soles and a hard floor is much higher than the friction between socks and a floor.
We call the measure of friction between two objects the coefficient of friction. We'll learn more about the coefficient of friction and how to calculate the force it can exert later, but for now, we just want to understand the basic concept: it varies based on the properties of the two objects coming into contact with one another
Real world examples are the best part of physics, so here are some common examples of friction:
- Tires – Like the bottoms of our sneakers, tires are made out of rubber for a reason: they have a high coefficient of friction with hard surfaces. We don't want our tires slipping on asphalt, otherwise, we'd be sliding around out of control. That definitely wouldn't be good for our driving record or car insurance rates.
- Brakes – Whether on a car or a bicycle, brakes use the concept of pressing brake pads onto a disc or drum with a resulting high coefficient of friction, thereby bringing us to a speedy halt.
- Wheel bearings – unlike tires and brakes, wheel bearings are designed to minimize friction. By using ball bearings that roll between two cylinders, we're able to keep the wheels rolling for a long time. This is why they're used on everything from semi-trucks to skateboards.
- Skipping rocks – Solids don't have a monopoly on friction. Liquids provide friction, too. That's why water slows down a skipping rock pretty quickly, dragging the poor rock to its watery grave. The same principle slows down boats, swimmers, baby squids, and anything else moving across or through water.
- Parachutes – That's right, the atmosphere is in on the friction game, too. We call the friction caused by an object moving through the atmosphere drag. Like friction, various properties affect the drag of an object. A parachute is designed to have high drag and thereby slow skydivers down. It's true, a parachute doesn't bring skydivers to a complete stop, but that's because gravity is also in play. In contrast to a parachute, a spare tire has very little drag. Good to keep in mind next time you're choosing between a parachute and a spare tire before jumping out of a plane.
Fight the Friction
As we can see in the diagram above, the force of friction is always in the opposite direction of the motion of the object (unless the object is not moving). This is what causes a negative acceleration for objects on the move, whether it's a plane flying through the air or Tom Cruise sliding on his socks.
This particular diagram also shows a "pushing force" in the same direction as the motion. This would represent the force necessary to counterbalance the force of friction and keep us moving along. In a car, the engine provides the moving force to not only get us moving from a stop but also to counteract friction and drag once we're up to speed. If it weren't for friction and drag, we'd be able to completely let off the gas pedal once we're on the freeway, and we'd keep rolling indefinitely. Or at least until we slammed on the brakes or ran into something.
The same goes for everything from a skateboard to a jumbo jet. With the skateboard, we use our legs to provide the pushing force to counteract friction and drag. A jumbo jet uses its engines to counteract drag. In fact, a jet is a great example of Newton's laws at work.
If a jet is flying at a constant speed in a straight line, then that means it meets the criteria of Newton's First Law of Motion. How is this possible with so many forces acting on it? Simple. The pushing force exerted by the jet engines is equal and opposite to the force of drag. (At the same time, the lift from the wings is equal and opposite to the force of gravity.) The net force exerted on the jet is zero, so it continues along at a constant velocity.
Back off on the jet engines and the force of friction becomes greater than the pushing force. We're now into the realm of Newton's Second Law and the jet experiences a negative acceleration. Crank the engines up higher and we get the opposite effect.
All of this comes into brilliant display if we go back to the example of skydiving. When we performed trajectory calculations in the previous unit, we assumed that the effect of gravity on an object is constant. In reality, that's not the case, thanks to the atmosphere and drag. As we discussed earlier, drag is dependent upon various factors. One of them is the velocity of the object. The faster an object moves, the greater the drag. This means if an object accelerates to a great enough velocity, the drag force will be equal and opposite to the force of gravity. All of a sudden the object will quit accelerating and travel at a constant velocity because it has a net zero force. Crazy, right?
This is what's known as terminal velocity. Every object has a different terminal velocity. A skydiver in standard belly flop position has a terminal velocity of around 200 km/hr, whereas a skydiver with an open parachute has a terminal velocity has a terminal velocity around 28 km/hr. That's a big difference, one that keeps skydivers from going splat on the ground.
Sample Lesson - Activity
Activity 5.02a: Lab – Skydiving With Coffee
Terminal velocity seems like a pretty weird concept at first. Can our flimsy little atmosphere really match up with the great big gravitational pull of the entire Earth? Well, considering that we overcome gravity every time we get out of bed, maybe it's not so hard.
We've learned that once an object is falling fast enough, the force of air resistance becomes equal and opposite to the force of gravity. At this point, the object will fall at a constant speed, since the forces on the object are balanced.
What determines an object's terminal velocity? Do heavy objects fall faster than lighter objects? Does the size of the object matter? What about the height it's dropped from? Would a bowling ball reach terminal velocity if we dropped it out of the window of our classroom?
To answer all these questions (except for maybe the last one), we'll go to the lab and approximate the very cool and dangerous sport of skydiving with a very safe and classroom-friendly alternative: coffee filters.
Materials you'll need to get together:
- Coffee filters (the bowl kind)
- Stopwatch
- Meter stick or measuring tape
- Tape
- Stepstool or chair to stand on
We'll be keeping track of all of our work in a Word document, so make sure to have that handy before we start.
Part One: What's going on here?
Coffee filters are useful because their large cross-sectional area and small mass allow them to reach terminal velocity quite quickly when they are released. But before we start dropping things, we need to figure out what forces are involved here.
Get out a blank piece of paper so that we can start doodling some force diagrams. You could also use Microsoft Paint or Power Point (or Paintbrush if you're on a Mac) and just insert your pictures into your Word doc when you're done. If you choose to draw on paper, you'll need to scan your paper or take a picture so that it can be uploaded with your Word doc at the end of the lesson.
Okay, back to the real science. We have three different situations to think about:
- The instant the filter is released, before it starts falling
- The beginning of the fall, before the filter has reached terminal velocity
- After the coffee filter has reached terminal velocity
For each of these situations, you'll need to draw a simple force diagram similar to the one in today's reading. The filter can be approximated as a box, or simply a dot if you prefer. Don't forget to label each diagram with the situation it belongs to.
The important information is in the arrows we draw, and the length of those arrows. Each arrow represents a force, and the length of the arrow represents how big that force is, which is important for visually showing the relative size of the forces involved. For example, our picture of Isaac Newton sitting at rest on a chair shows that the normal force of the chair pushing up is equal to the force of gravity pulling him down. We know that the two forces are equal because the arrows are roughly the same length.
Hey, we've seen this somewhere before…
So what force(s) are actually acting on the coffee filter? We know that gravity is always a factor. We also know that when an object isn't moving, there isn't any air resistance. Lastly, when an object is moving at a constant velocity, the forces must be balanced. Armed with these facts, go ahead and make those force diagrams before moving onto the next step.
Part Two: Measuring Terminal Velocity
Measuring terminal velocity sounds hard. The coffee filter looks like it's falling at a constant velocity, but how do we really know? Time to get back to the basics: velocity is really just displacement divided by time. We knew that, like, months ago. By timing how long it takes the coffee filter to fall a certain distance, we can calculate its velocity.
We'll take data for a few different situations and then analyze it at the end. Time to whip out the stopwatches and go to work.
Using tape, mark 0.5 meter increments on the wall, starting from the floor and going up to 3 meters. Alternatively, if you have three meter sticks, you can just tape them all to the wall stacked on top of one another, and then leave it up as a decoration when you're done. We'll just call it modern art.
Drop a coffee filter from the 2 m mark and time how long it takes to fall just the first meter. Do it again, this time timing how long it takes to fall just the second meter. Take each of these measurements three times total and record your results in a table in your Word doc that looks like the one below.
Part Three: Does height matter?
Drop the coffee filter from at least three different heights higher than 1.5 m, measuring only the time it takes the filter to fall the last meter before it hits the floor. Repeat the experiment for each height three times and record the data in a (titled) table in your Word doc.
Part Four: Do heavier skydivers fall faster?
Coffee filters are cool because they are stackable, effectively allowing us to double the mass without changing the surface area. Also because they make delicious coffee.
Again drop the coffee filter from 2 m, measuring only the time it takes to fall the last meter before it hits the floor. Then, stack two filters together and repeat the measurement. Do this for three stacked filters as well, recording all of the data in a table in your Word doc.
Part Five: Post Lab Questions
Okay, we've dropped a lot of coffee filters, and maybe even drunk some coffee, but what does it all mean?
First, use the data from part four to calculate the terminal velocity of the stacked coffee filters. Record the results in a new table in your Word doc.
Then, answer the questions below in your Word doc using complete sentences. Where force diagrams are needed, you can either draw on paper and upload an appropriately labeled picture, or insert computer-generated images into your Word doc.
1. Why aren't all of the trials for each situation exactly the same? Describe at least three possible sources of error in this experiment as well as how those sources of error might affect your results. (150-200 words)
2. Did the single coffee filter fall faster in the first half or second half of its fall? Explain your answer using data from part two. Why? What was its terminal velocity? (150-200 words)
3. Does terminal velocity depend on the height that the coffee filter is dropped from? Explain your answer using data from part three. In addition, explain your answer using Newton's First Law. (150-200 words)
4. What happens to the terminal velocity as the mass (i.e. number of filters) increases? Use force diagrams to explain the physics behind this result. (200-300 words)
5. At a certain speed, objects with a smaller cross-sectional area will have less drag than objects with a larger cross-sectional area. If you were to drop a smaller coffee filter and a larger coffee filter of the same mass, which one would have a greater terminal velocity? Explain your reasoning using force diagrams. (200-300 words).
Upload your Word document below. Be sure to include your force diagrams from part one.
Lab Report Rubric - 50 Points
Sample Lesson - Activity
- Credit Recovery Enabled
- Course Length: 18 weeks
- Course Type: Basic
- Category:
- Science
- High School
Schools and Districts: We offer customized programs that won't break the bank. Get a quote.
